Forming and Producing New Neurons
- Reviewed9 Jan 2023
- Author Lindzi Wessel
- Source BrainFacts/SfN
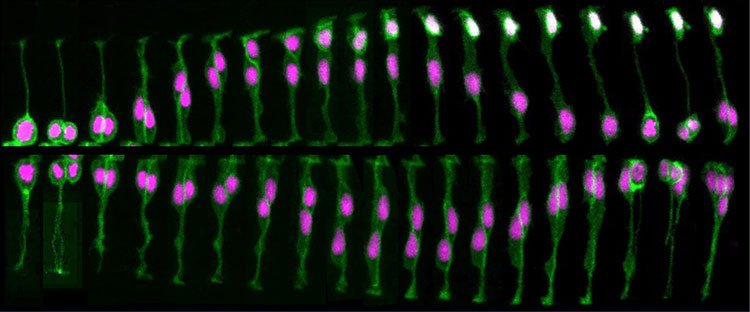
Neurons develop through delicate and carefully choreographed processes that take place while an embryo grows. Signaling molecules “turn on” certain genes and “turn off” others, initiating the formation of immature nerve cells. During the next stage — cell division, also called proliferation — the pool of early-stage brain cells increases by billions. Finally, during migration, these newly formed neurons travel to their final destinations. The nervous system formed by these processes is active throughout life, making new connections and fine-tuning the way messages are sent and received.
Formation and Induction
During the very early stages of embryonic development, three layers emerge — the ectoderm (outer-most layer), mesoderm (middle layer), and endoderm (inner-most layer). Although the cells in each layer contain identical DNA instructions for development, these layers ultimately give rise to the rich variety of tissue types that make up the human body. The explanation for this diversity lies in signals produced by surrounding tissues. Those signals turn certain genes on and others off, thus inducing the development of specific cell types. Signals from the mesoderm trigger some ectoderm cells to become nerve tissue, a process called neural induction. Subsequent signaling interactions refine the nerve tissue into the basic categories of neurons or glia (support cells), and then into subclasses of each cell type.
The fate of a developing cell is largely determined by its proximity to various sources of signaling molecules. The concentration of each type of signaling molecule decreases farther from its source, creating gradients throughout the brain. For example, a particular signaling molecule, called sonic hedgehog, is secreted from mesodermal tissue lying beneath the developing spinal cord. As a result of exposure to this signal, adjacent nerve cells are converted into a specialized class of glia. Cells that are farther away are exposed to lower concentrations of sonic hedgehog, so they become motor neurons that control the movement of muscles. An even lower concentration promotes the formation of interneurons, which don’t relay messages to muscles but to other neurons. The mechanism of this molecular signaling is very similar in species as diverse as flies and humans.
Proliferation
In the brain, neurons arise from a fairly small pool of neural stem and progenitor cells, special cells that can divide and become a variety of mature cell types. Before achieving their mature cell fate, this pool of cells undergoes a series of divisions — increasing the number of cells that will ultimately form the brain. Early divisions are symmetric — the split results in two identical daughter cells, both able to keep dividing. But as these divisions progress, the cells begin to divide asymmetrically, giving rise to only one daughter cell that keeps proliferating and a second that progresses towards its ultimate cell fate as a neural or glial cell (the exact sequences and ultimate fates vary by species).
This proliferative process permits rapid growth during early development of the brain, with billions of cells being produced in a matter of weeks. After that series of divisions is complete, only a few neural stem and progenitor cells remain within the brain, and neurogenesis in adulthood is limited to a few regions of the brain, such as those involved with memory.
Scientists have proposed that protein defects causing a premature switch from symmetric to asymmetric divisions may be a cause of microcephaly. This disorder, characterized by a severe reduction in brain size, is associated with serious neurological disabilities and sometimes death in infancy. Similarly, excessive proliferation of brain cells can lead to a disorder called megalencephaly — a brain that is abnormally large and heavy — which is also associated with a variety of neurodevelopmental complications.
Adapted from the 8th edition of Brain Facts by Lindzi Wessel.
CONTENT PROVIDED BY
BrainFacts/SfN
References
Clarke, L. E., & Barres, B. A. (2013). Emerging roles of astrocytes in neural circuit development. Nature Reviews Neuroscience, 14(5), 311-321. doi:10.1038/nrn3484
Dabrowski, A., Johnson-Venkatesh, E., & Umemori, H. (2017). Synaptic Development. Conns Translational Neuroscience, 89-111. doi:10.1016/b978-0-12-802381-5.00006-3
Ganguly, K., & Poo, M. (2013). Activity-Dependent Neural Plasticity from Bench to Bedside. Neuron, 80(3), 729-741. doi:10.1016/j.neuron.2013.10.028
Homem, C. C., Repic, M., & Knoblich, J. A. (2015). Proliferation control in neural stem and progenitor cells. Nature Reviews Neuroscience, 16(11), 647-659. doi:10.1038/nrn4021
Kolb, B., & Gibb, R. (2014). Searching for the principles of brain plasticity and behavior. Cortex, 58, 251-260. doi:10.1016/j.cortex.2013.11.012
Lenroot, R. K., & Giedd, J. N. (2006). Brain development in children and adolescents: Insights from anatomical magnetic resonance imaging. Neuroscience & Biobehavioral Reviews, 30(6), 718-729. doi:10.1016/j.neubiorev.2006.06.001
Noctor, S., Cunningham, C., & Kriegstein, A. (2013). Radial Migration in the Developing Cerebral Cortex. Cellular Migration and Formation of Neuronal Connections, 299-316. doi:10.1016/b978-0-12-397266-8.00027-2
Ozair, M. Z., Kintner, C., & Brivanlou, A. H. (2012). Neural induction and early patterning in vertebrates. Wiley Interdisciplinary Reviews: Developmental Biology, 2(4), 479-498. doi:10.1002/wdev.90
Perrimon, N., Pitsouli, C., & Shilo, B. (2012). Signaling Mechanisms Controlling Cell Fate and Embryonic Patterning. Cold Spring Harbor Perspectives in Biology,4(8). doi:10.1101/cshperspect.a005975
Plasticity. (2012, April 1). Brainfacts.org. Retrieved from: http://www.brainfacts.org/brain-basics/brain-development/articles/2012/plasticity/
Pollard, T. (2015). New Light on Growth Cone Navigation. Developmental Cell, 35(6), 672-673. doi:10.1016/j.devcel.2015.12.002
Sala, C., & Segal, M. (2014). Dendritic Spines: The Locus of Structural and Functional Plasticity. Physiological Reviews, 94(1), 141-188. doi:10.1152/physrev.00012.2013
Sorg, B. A., Berretta, S., Blacktop, J. M., Fawcett, J. W., Kitagawa, H., Kwok, J. C., & Miquel, M. (2016). Casting a Wide Net: Role of Perineuronal Nets in Neural Plasticity. The Journal of Neuroscience, 36(45), 11459-11468. doi:10.1523/jneurosci.2351-16.2016
Sun, G. J., Zhou, Y., Stadel, R. P., Moss, J., Yong, J. H., Ito, S., Kawasaki, N. K., Phan, A. T., Oh, J. H., Modak, N., et al. (2015). Tangential migration of neuronal precursors of glutamatergic neurons in the adult mammalian brain. Proc. Natl. Acad. Sci., 112, 9484–9489. doi:10.1073/pnas.1508545112
Werker, J. F., & Hensch, T. K. (2015). Critical Periods in Speech Perception: New Directions. Annual Review of Psychology, 66(1), 173-196. doi:10.1146/annurev-psych-010814-015104
What to Read Next
Also In Brain Development
Trending
Popular articles on BrainFacts.org